Photosynthetic Signatures (PS)
- Size of the PSII-associated light harvesting pigments, or more precisely, by the total number of chlorophyll molecules serving PSII reaction center. This property is generally referred to as the "optical absorption cross section of PSII", aPSII.
- Spectral composition of the light harvesting pigments. Generally, σ PSII is wavelength-dependent.
- Efficiency of excitation transfer from pigment bed to PSII reaction center, θ tr. This property is controlled by the architecture and organization of diverse group of Light Harvesting Complexes, by the phosphorylation status of some of these complexes, and by the epoxidation status of some carotenoids within these complexes.
- Yield of Charge Separation in Photosystem II reaction center Φ PSII: a ratio of stable charge separation events to number of excitation quanta arriving at the reaction center.
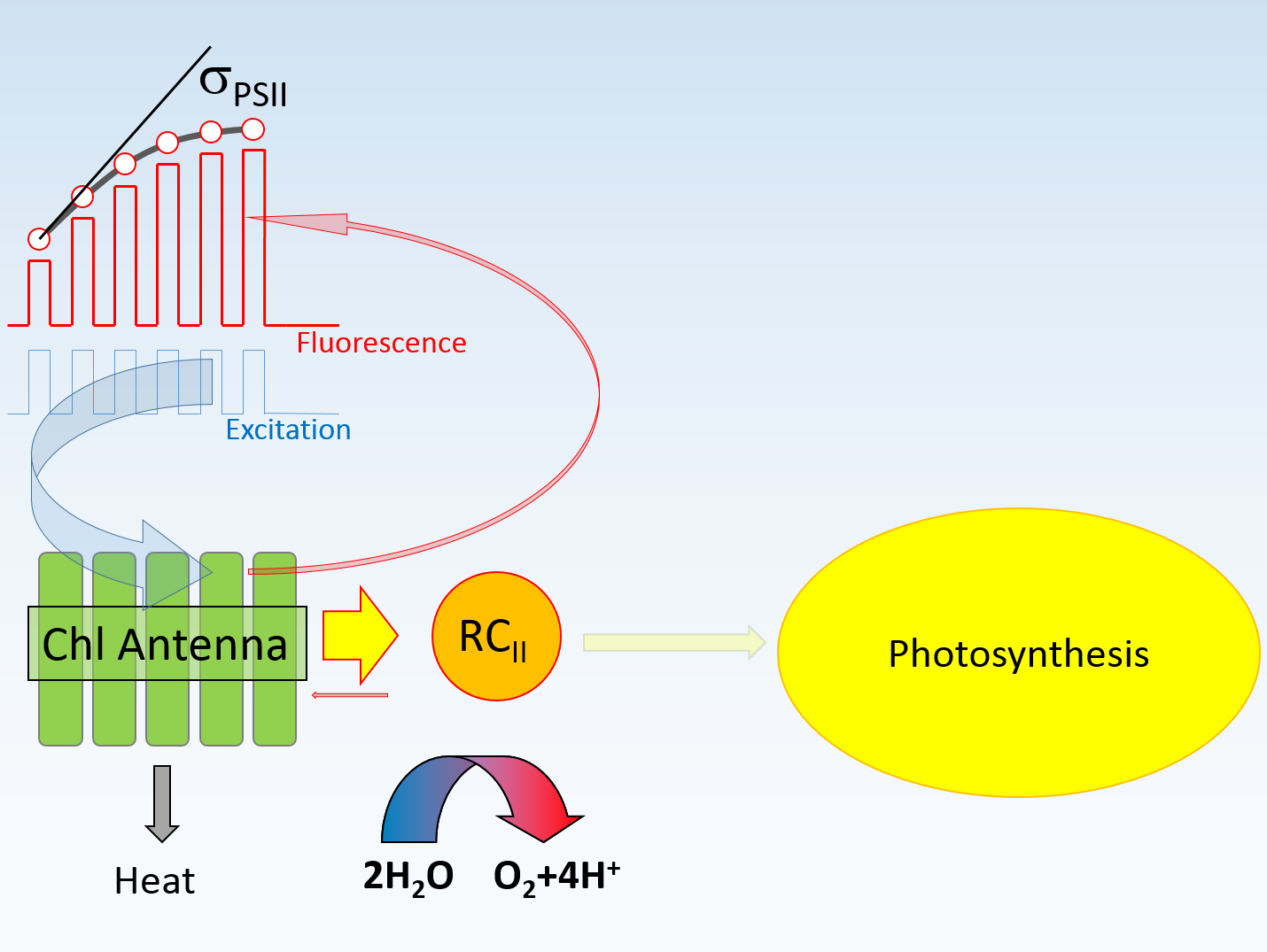
Theoretically, functional absorption cross section
can be estimated from the rate of charge separation normalized to quanta flux under conditions of low illumination level.
Because rates of charge separation are difficult to measure under steady-state illumination, the excitation signal in LIFT technique is produced as series of high-frequency flashlets (see the previous section),
and σ PSII is estimated from the curvature of the observed fluorescence transients. This parameter can be approximated, to a first degree, from the
initial slope of fluorescence transient versus excitation energy, normalized to variable fluorescence, Fv.
This approximation, however, is only valid under conditions of blocked electron transport between PSII and PSI, and in absence of energy transfer between PSII units.
These conditions cannot be satisfied in vivo.
Instead, we calculate the σ PSII by fitting the
entire fluorescence transient
to a model described by Kolber et. al, 1998. This approach provides simultaneous assessment of the kinetics of the electron transport between PSII and PSI,
and quantifies the extent of energy transfer between PSII units. Here are the examples of actual fluorescence transient acquired with marine phytoplankton displaying
σ PSII = 423 A2/RCII and
σ PSII = 786 A2/RCII, respectively.
The initial slope of the fluorescence transient is "roughly" proportional to the calculated functional absorption cross section.
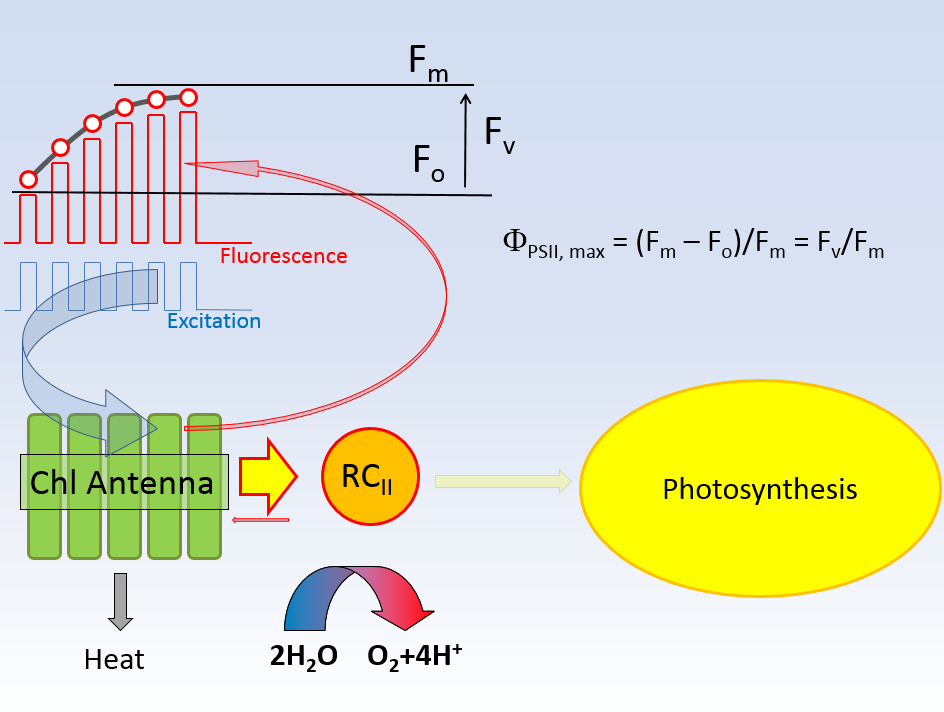
The yield of charge separation attains a maximum value, Φ PSII, max, when the initial level of the fluorescence signal, Fo, is measured
under conditions of fully oxidized QA, and Fm signal is measured under conditions of fully reduced QA. These conditions cannot be met experimentally:
measurement of the fluorescence requires application of an excitation signal, invariably resulting in some level of QA reduction. Also, full reduction of QA is
unattainable in vivo because of a finite level of electron transport from QA to PSII. Nevertheless, applying low-intensity excitation to measure Fo,
and strong excitation signal to measure Fm, quite good approximation of the true Fo and Fm signals can be obtained.
In the LIFT/FRR method, both these signals are calculated by fitting the fluorescence transient to a model describing the relationship between
fluorescence and excitation signal, thus avoiding the problem of "approximate" assessment. The quality of this approach, however, depends on the quality
of the models, which also have some limitations.
Another problem is related to the experimental protocols used to perform reduction of QA.
Using a QA flash, a high intensity excitation beam is used
to reduce QA within 200-800 µs, with negligible electron outflow to PQ pool. In another protocol,
a PQ flash with much lower excitation power
is applied over longer period of time to progressively reduce PQ pool, with subsequent QA reduction(1). Although these two protocols should reduce QA,
there is another factor modulating the maximum level of fluorescence signal, independent of QA reduction. I has been argued that high excitation energy used in the
QA flash prevents the fluorescence signal from reaching the maximum level due to the "donor side quenching".
In our opinion this notion is incorrect. Rather, it is the level of PQ pool reduction (or more precisely the level of QB reduction) that
determines the final amplitude of the maximum fluorescence yield. To demonstrate that, we apply
a sequence of QA and PQ flash in presence of 20 µE of IR (730 nm) light.
Immediately, the Fv/Fm in the QA flash decreases to a value 0.66, while the PQ-flash signal rises, in proportion to PQ pool level reduction,
to Fv/Fm of 0.82. When a
pair of two ST flashes is applied following ~1 minute of dark adaptation,
the first one generally displays ~25% higher fluorescence yield.
As PSI becomes engaged during the relaxation part of this flash, PQ pool reoxidizes, and the maximum fluorescence yield attainable during second QA flash decreases.
Again, when these two flashes are
applied in a presence of ~ 20 µE of IR (730 nm) light,
the fluorescence yield in both flashes becomes equal, with Fv/Fm of 0.63.
These observations also indicate that the PQ pool in plants becomes significantly reduced within several minutes in darkness.
Although it is possible, using appropriate excitation protocols, to push the Fv/Fm signal to a level of 0.9 and beyond,
the utility of such exercise appears to be of little value in assessing the "correct" value of Φ PSII, max.
Much more interesting question is what controls the variability of the Fm signal, what information it provides,
and how this control is affected by the environmental conditions. The LIFT/FRR technique, by operating with QA flashes and PQ flashes allows addressing these questions.
This effort, however, may be of little relevance for assessing Φ PSII under conditions of engaged electron transport between PSII and PSI.
(1)The QA and PQ flashes were earlier referred in the literature as single-turnover (ST) flash and multiple-turnover (MT) flash. While the definitions of
PQ and MT flash are equivalent, the practical implementation of the QA flash generally produce a little bit more than a single electron per PSII reaction center. To avoid confusion, we use the term "QA flash"
to indicate excitation condition resulting in reduction of QA with minimal (less than 10%) electron transfer to the PQ pool.
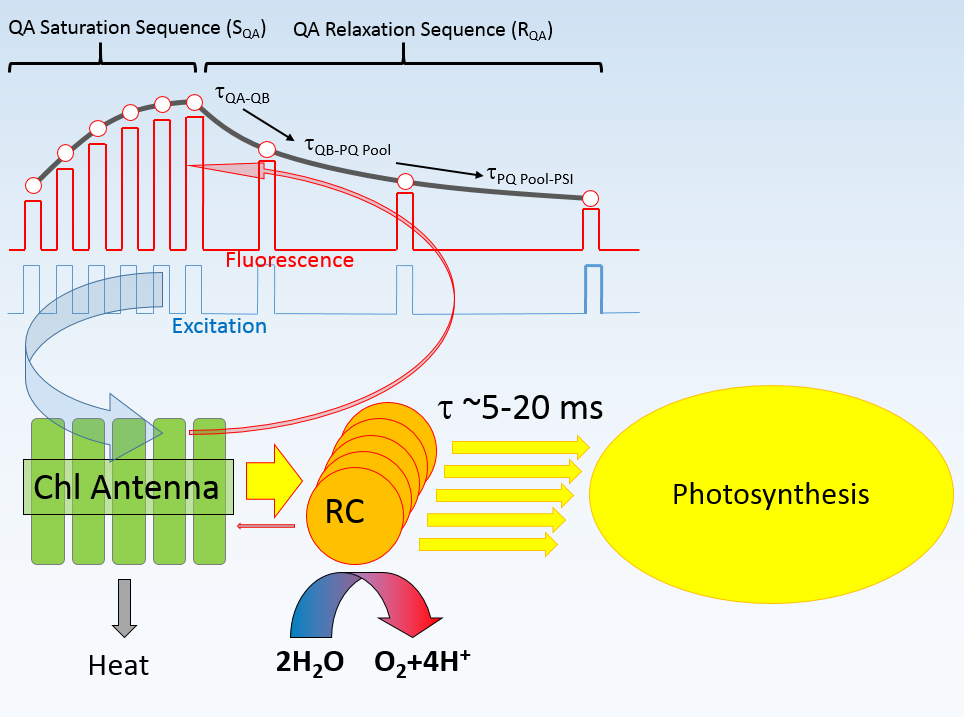
Once the SQA portion of the excitation protocol is completed, the time interval between flashlets is increased in an exponential fashion to observe the kinetics of QA reoxidation (the RQA portion of QA flash). This kinetics defines the shape of the fluorescence relaxation curve, and can usually be expressed as a sum of two, three, of four exponential components. This interpretation, however, doesn't reflect the biophysical nature of the electron transport phenomenon. Instead, we express this kinetics in terms of a multistage, sequential electron transport: QA → QB, QB → PQ pool, and PQ pool → PSI. We assume that two electrons can be stored on QB, and we allow the software to calculate the actual size of the oxidized portion of PQ pool. We allow for the back-reaction between QB and the donor side of PSII, generally with time constant of about 300 ms, and calculate the amplitude of the hypothetical charge recombination signal, assumed to be proportional to the level of QB reduction. As there is a number of free parameters in our fitting procedure, a question arises regarding the robustness of the analysis with so many degrees of freedom. To address this question, the user have a choice of including/excluding any of these parameters from the analysis, or fixing any of these parameters at an arbitrary level, thus reducing the degree of freedom in the fitting procedure. The quality of the fit can then be judged from the χ2 measure, and from the graphical representation of the fit residuals. Here we present an example of data analysis using high-quality fluorescence transient (S/N > 3000), at varying levels of degree of freedom.
- Assume single-stage electron transfer
between PSII and PSI.
Although the time constant of this transfer, about 5 ms, is reasonable, the quality of the fit, judged from high χ2 =2964, and from large residuals, is inadequate. The purple trace in the plot show the calculated level of QA reduction. - Assume double-stage (QA → PQ pool → PSI
electron transfer.
The fit quality is a little bit better, with χ2 = 1034, and slightly better distribution of residuals. The brown trace display the calculated level of PQ pool reduction. - Assume three stage, QA → QB→ PQ Pool→ PSI
electron transport model.
The quality of the fit increased dramatically (χ2 =48.7), with much better distribution of residuals. Nevertheless, closer analysis of residuals reveals that the model has some problems at the initial stage of the saturation sequence, and in the relaxation portion (Remember that the residuals are amplified by a factor of 5) - Allow for back reaction.
There is small improvement in the fit quality (χ2 = 32.1), but distribution of residual remains similar. - Make the probability of energy transfer a free parameter in the fit.
There is virtually no change in the quality of the fit, indicating that the assumed arbitrary vale of 0.2 was a quite good guess for this parameter. - Allow calculation of charge recombination between QB and the donor side.
The value of χ2 decreased to 21.9, with slight improvement of the residuals distribution. Adding other parameter to the fit (donor side quenching, making the time constant of back-reaction free, changing the initial level of PQ pool reduction) didn't improve the quality of the fit. A relatively high value of χ2 indicates that our model, not the quality of data, limits the quality of the fit.
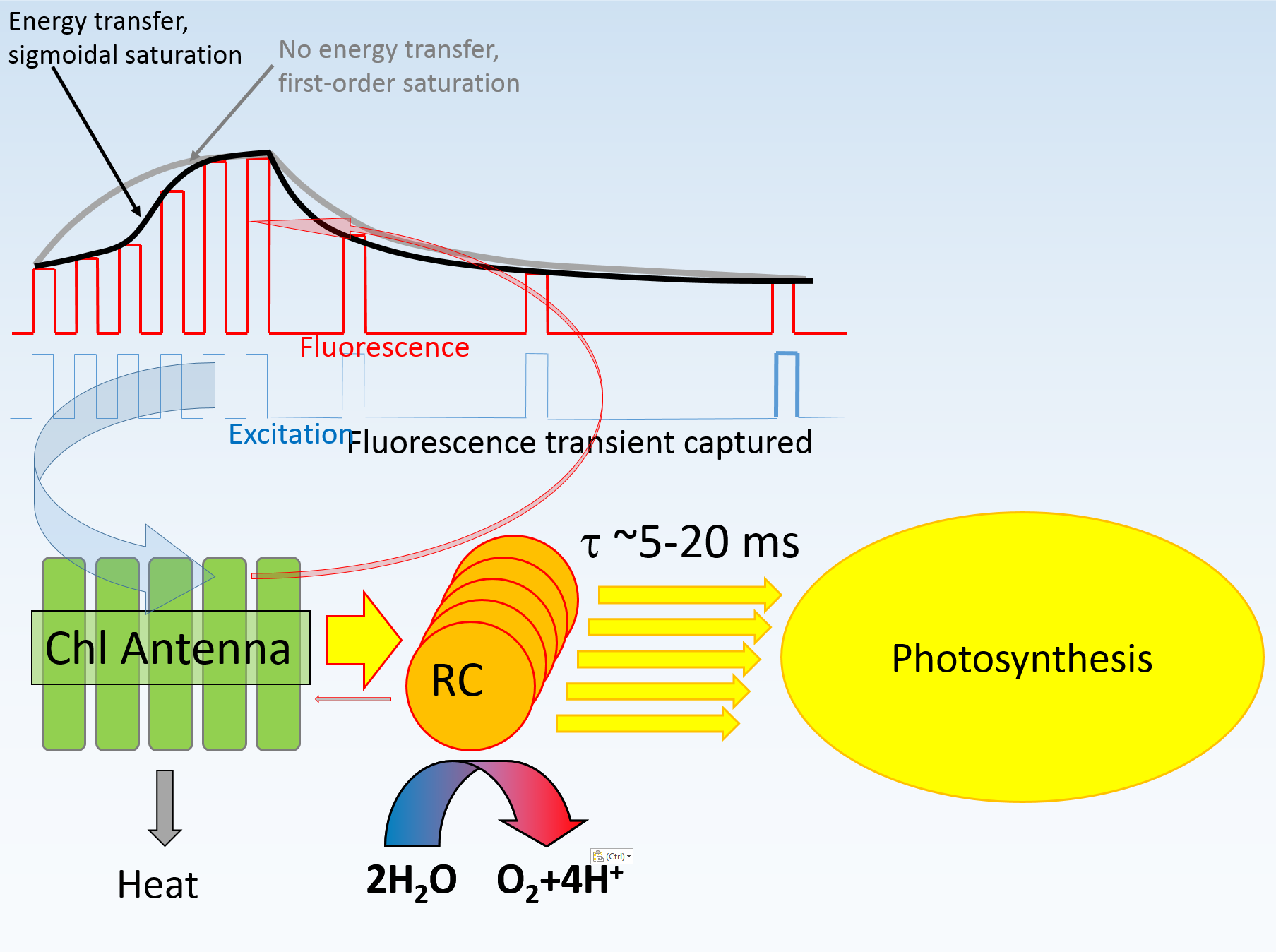
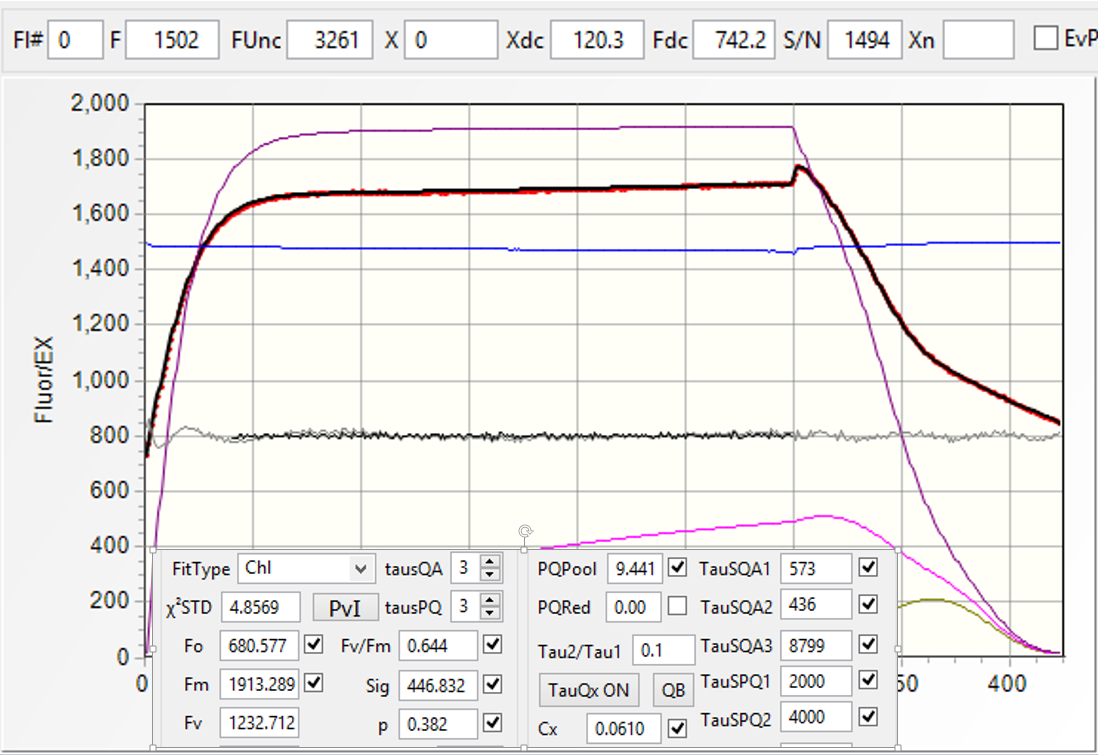
The level of PQ pool reduction can easily be manipulated by application of ambient illumination. By the same token, FRR excitation signal will also modify PQox (the entity to be measured). To assess this effect, a sequence of 12 FRR flashes was applied at 1 second interval on IR pre-illuminated lemon tree leaf with PQox quantified at each flash. Indeed, the measured PQox decreased from 6.97 to 3.1, with about 3% decrease after the first flash. Therefore, assessment of PQox should be performed using QA flash with as little excitation energy as practically possible to minimize the flash-induced inflow of electrons to the PQ pool. When LIFT-FRR flashes are applied in a quick sequence under conditions of low (less that 1 μmol quanta m-2s-1 of white/IR light), the amplitude of fluorescence signal progressively increases, roughly in proportion to the level of PQ pool reduction (see the Charge Separation Section above). Ultimately, at close-to-full PQ pool reduction level, the amplitude of the measured fluorescence signal approaches that observed in the presence of DCMU. Because in the latter case the PQ pool reduction relevel should remain low, it is hypthesized that the occupancy level of QB site on D1 protein is the ultimate factor controlling the observed changes in the fluorescence signal. With increasing level of PQ pool reduction the quinone/hydroquinone equilibrium in the PQ pool shifts toward the latter, the fraction of un-occupied QB sites decreases, the electron residency time on QA increases, and the fluorescence yield increases to a level observed in presence of DCMU. Although the mechanisms by which the QB site un-occupancy (or electron residency time on QA) produces up to 40% increase in the fluorescence signal (up to 100% increase in terrestrial plants) remains unclear, it is likely that this mechanisms is also responsible for modulation of fluorescence signal during PQ flash compared to that of the QA flash (see the section on excitation protocols in the LIFT-Terrestrial section).
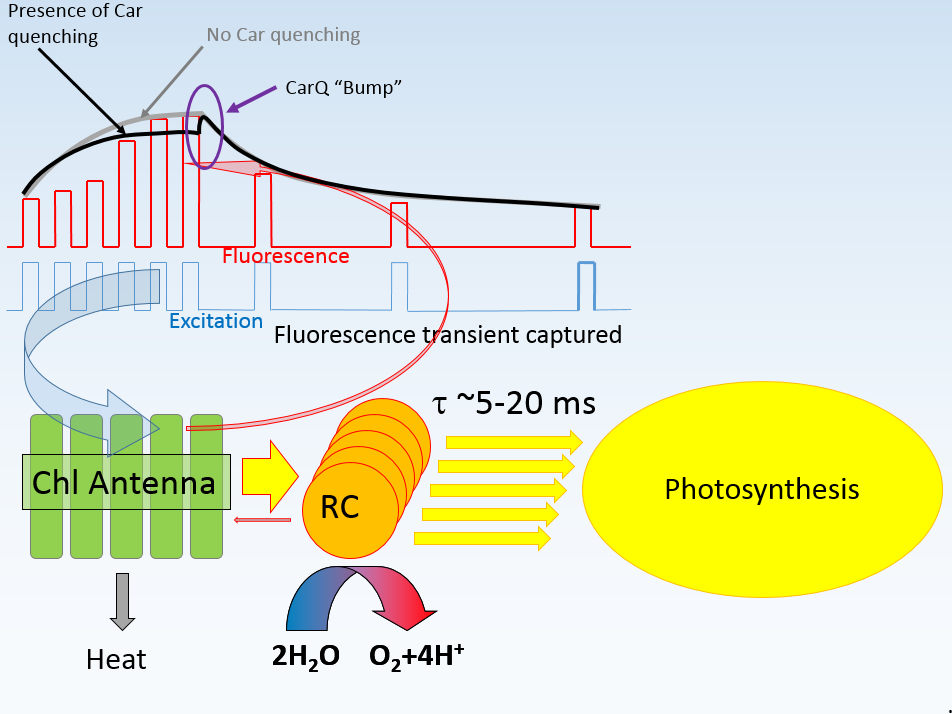
The CarQ is characterized by two parameters: the time constant of dissipation (in the transient shown, it equals 18 µs), and by the amplitude relative to the excitation power. LIFT-FRR software allows CarQ fitting using three different models:
- Assuming that the amplitude of CarQ is controlled by the excitation power alone
- Assuming that the amplitude of CarQ is controlled by the level of QA reduction
- Assuming that the amplitude of CarQ is controlled by the product of excitation power and QA reduction level
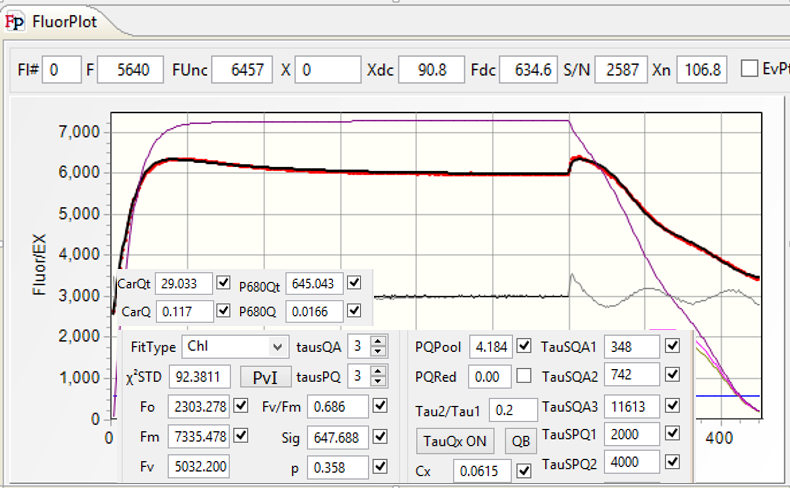
NOTE: Observation of the donor side quenching in leaves of higher plants requires average excitation power of ~30,000 to 50,000 µmol quanta m-2s -1. In marine phytoplankton this quenching is observed at much lower excitation power due to average 2-3 times higher functional absorption cross section.